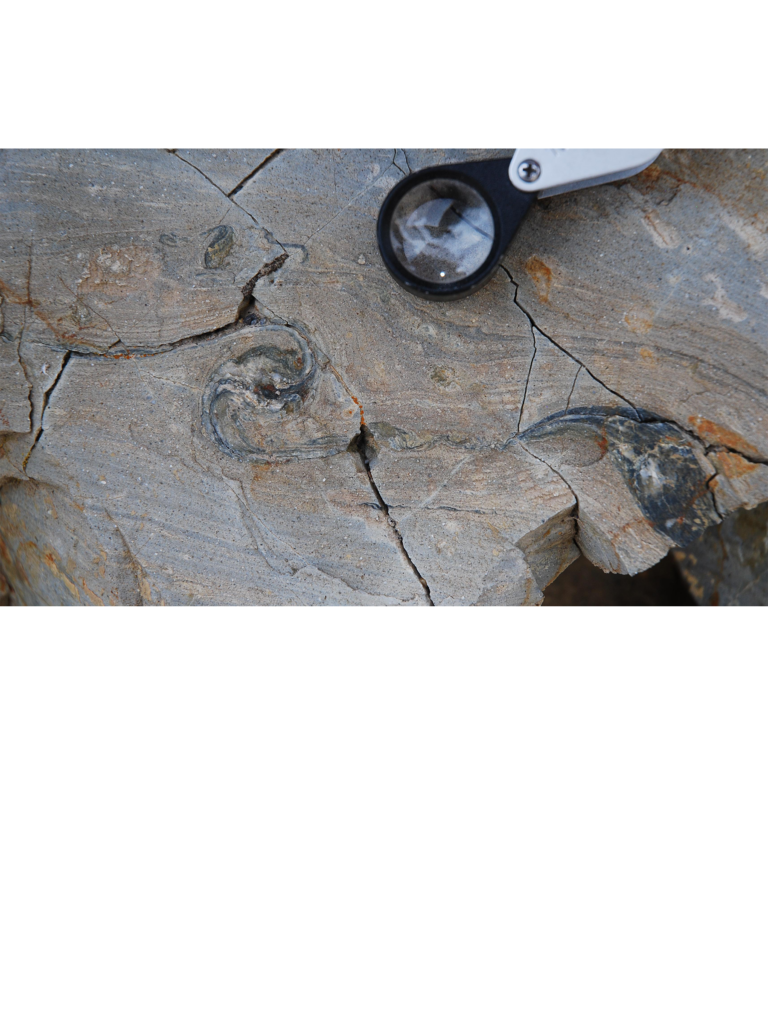
Deep structure
Can we predict the width, structure, and rheology of large scale faults at depth within the Earth?
We propose three concepts that allow us to do this:
- Ductile shear zones at any given depth operate at approximately constant stress.
- The variation of stress and temperature (and hence depth) through the crust in exhumed shear zones can be determined from the microstructure and mineral compositions.
- Shear zone rheology, and hence the degree of strain localization, can be calculated from the microstructure and from mineral flow laws.
If shear zones operate at constant stress, stress-temperature profiles from shear zones are proxies for the strength of the lithosphere. Grain-size reduction at constant stress will result in a switch in rheology to grain-size sensitive creep, causing strain localization. Rheology and hence strain rate can be predicted from flow laws for grain-size sensitive creep. From the strain rate we can calculate the width of a shear zone required to accommodate the plate-boundary displacement rate. John Platt, Alex Lusk, and Tarryn Cawood are currently collaborating on this project.
About the Image
Ultramylonites like this one pictured from the Ruby Mtns detachment zone can accommodate very high strains and strain-rates due to grain-size sensitive creep, which means they form relatively narrow shear zones (tens to hundreds of meters). Rocks deforming by dislocation creep, for example, cannot accommodate such high strain-rates, and form much broader shear zones (kms to tens of kms).
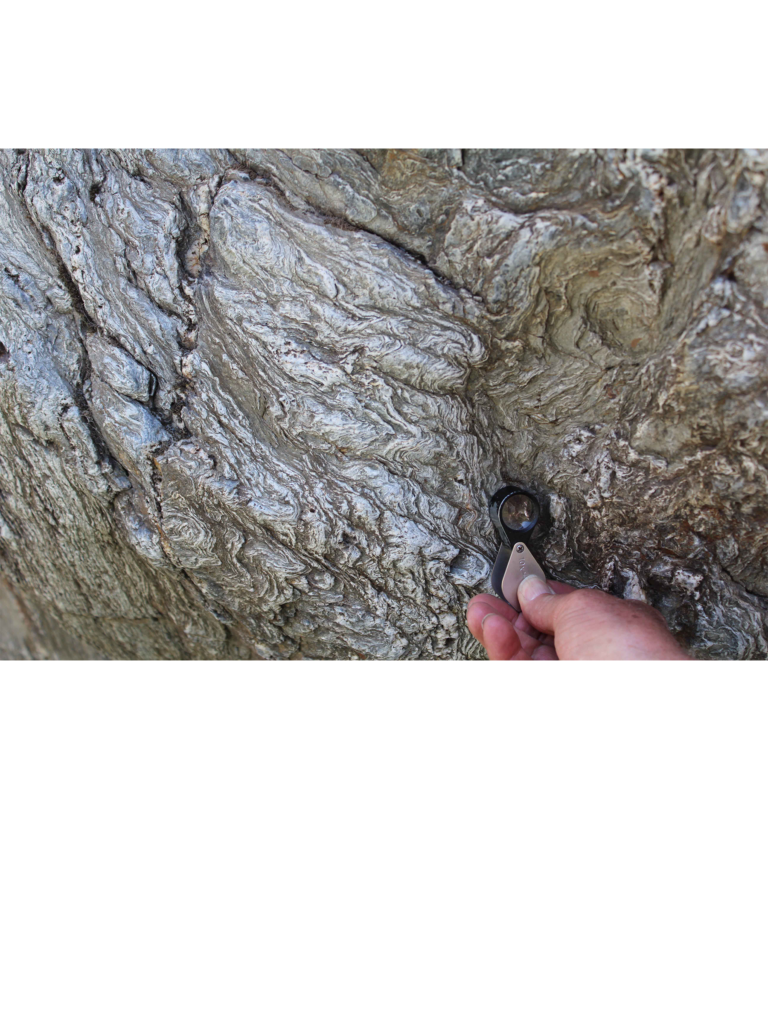
Geological constraints on the physical state of the subduction
The most powerful and damaging earthquakes known occur between 15 and 40 km depth along subduction zone interfaces. The down-dip end of this interval marks the transition from seismic slip to aseismic creep or ductile flow, and is also the source area for episodic seismic tremor and slow-slip (ETS) events. This transition may be instrumental in the loading of the seismically active section above it, and hence play a role in the triggering, and possibly the down-dip arrest, of seismic events. It is therefore of great interest and importance to establish the physical conditions at this depth, the structure and mechanical properties of the rock materials that accommodate the strain, and the nature of the creep and aseismic slip processes that produce ETS events.
The South Fork Mountain Schist (SFMS), which forms part of the Franciscan subduction complex in the Northern Coast Ranges of California, is a 240 km long and up to 3.5 km thick body of intensely deformed and metamorphosed sedimentary and volcanic rock that occupied the subduction zone interface in Early Cretaceous time. The depth and temperature of metamorphism are comparable to the source area of present-day ETS events. The SFMS preserves an extraordinary high-strain deformational microstructure that indicates the activity of a combination of microcracking, pressure-solution and dislocation creep at relatively high stress, burial depths of around 40 km, and temperatures of around 350°C. The SFMS offers an easily accessible window into the physical conditions and deformational mechanics during ETS events. Will Schmidt and John Platt have been quantifying (a) deviatoric stress using recrystallized grain-size piezometry on rocks affected by dislocation creep, (b) strain and strain-rate using the estimated subduction rate for this period, the thickness of the deformation zone, and microstructural features including microfold geometry and deformed clastic quartz grains, (c) temperature using laser Raman spectroscopy on carbonaceous material, and (d) pressure (and hence depth) using the Si content of white mica.
About the Image
Microfolds in South Fork Mountain Schist. The folded foliation is an intensely differentiated fabric produced by pressure solution during high strain deformation in the Franciscan subduction zone. This fabric is microfolded, and a new cleavage is developing along the limbs of the microfolds. The South Fork Mountain Schist forms a zone up to 3.5 km thick of intense strain that formed immediately below the Early Cretaceous subduction zone interface.
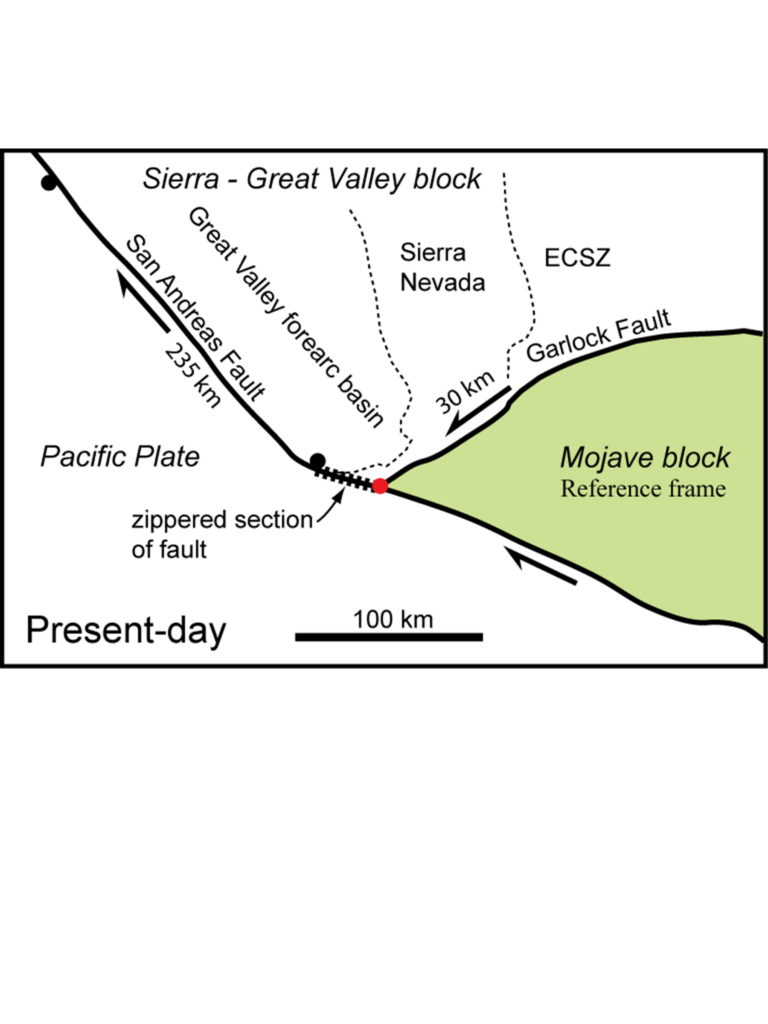
Intersecting conjugate faults
In many places around the world, a strike-slip fault with one sense of slip intersects another with the opposite sense of slip at a high angle. An example is the San Andreas fault, which slips to the right, and intersects the Garlock fault, which slips to the left, ~150 km north of Los Angeles. These intersections present a problem – if one fault cuts and offsets the other, it should deactivate it, yet in many cases both faults are known to be active. The faults commonly appear to merge without offset, even if they have displacements of tens or hundreds of km. This requires considerable distortion and rotation of the surrounding crust. Cees Passchier and I have proposed that the two faults may zipper up, merging into one fault, so that crust from one side of one of the faults is juxtaposed against crust from the opposite side of the other fault. This concept may explain a number of similarly enigmatic relationships, such as the intersection of the North and East Anatolian faults, the Giudicarie and Tonale faults in the Alps, and the Karakoram and Altyn Tagh faults in the eastern Pamirs.
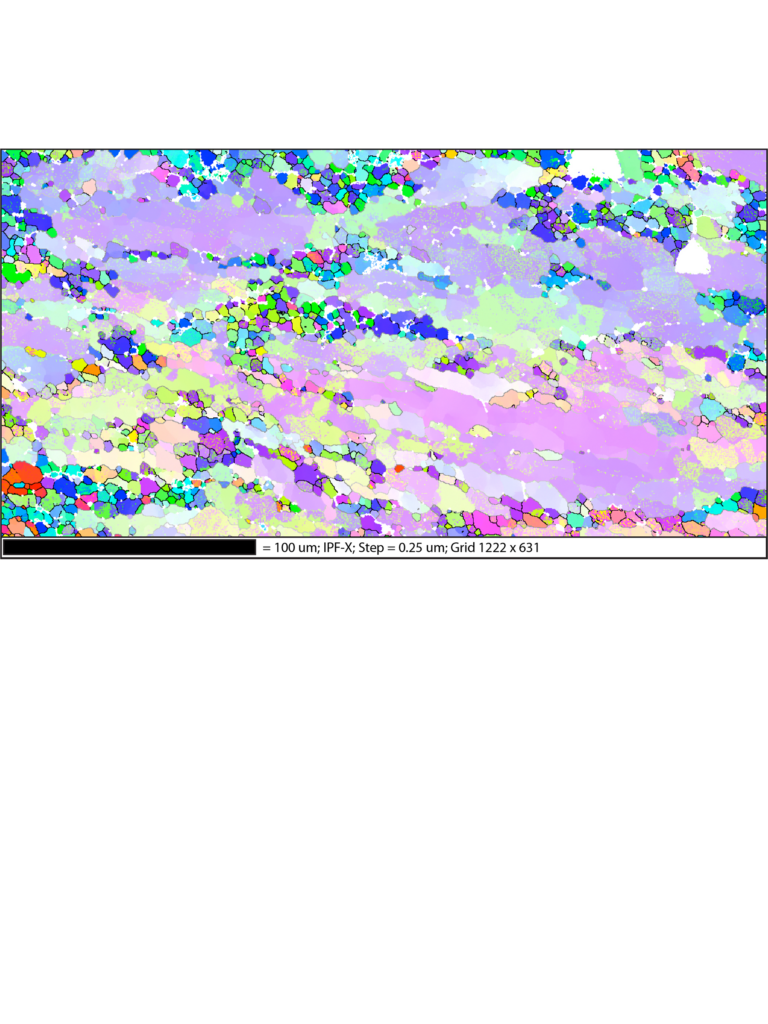
Dynamic recrystallization
At high stresses and low temperatures, grain-size reduction by dynamic recrystallization profoundly modifies rock rheology. John Platt, Whitney Behr, and Haoran Xia, in collaboration with Hans de Bresser, Tom Mitchell, and Dave Prior, have been investigating the mechanisms of grain-size reduction and the controls on the resulting grain size. This work has implications for the rheology of rocks deforming by crystal plasticity, for the mechanisms of strain localization, and for recrystallized grain-size piezometry. Recent and current work includes investigation of the stress sensitivity of microstructures such as subgrains and grain-boundary bulges in experimentally deformed calcite, the extent of post-nucleation grain growth in quartz undergoing recrystallization by grain-boundary bulging, and experimental work on high-temperature, low-stress microstructures in ice.
About the Image
The image shows an EBSD map of dynamically recrystallized quartz from the Whipple Mtns metamorphic core complex. Large purple grains are relict from an earlier high-T microstructure, these have been deformed and show subgrains and recrystallization by both subgrain rotation and grain-boundary bulging. The matrix shows a greater variation in crystal orientations due to continued crystal-plastic deformation; strain-energy-driven grain-boundary migration has also continued, creating curved, lobate, and serrated boundaries.
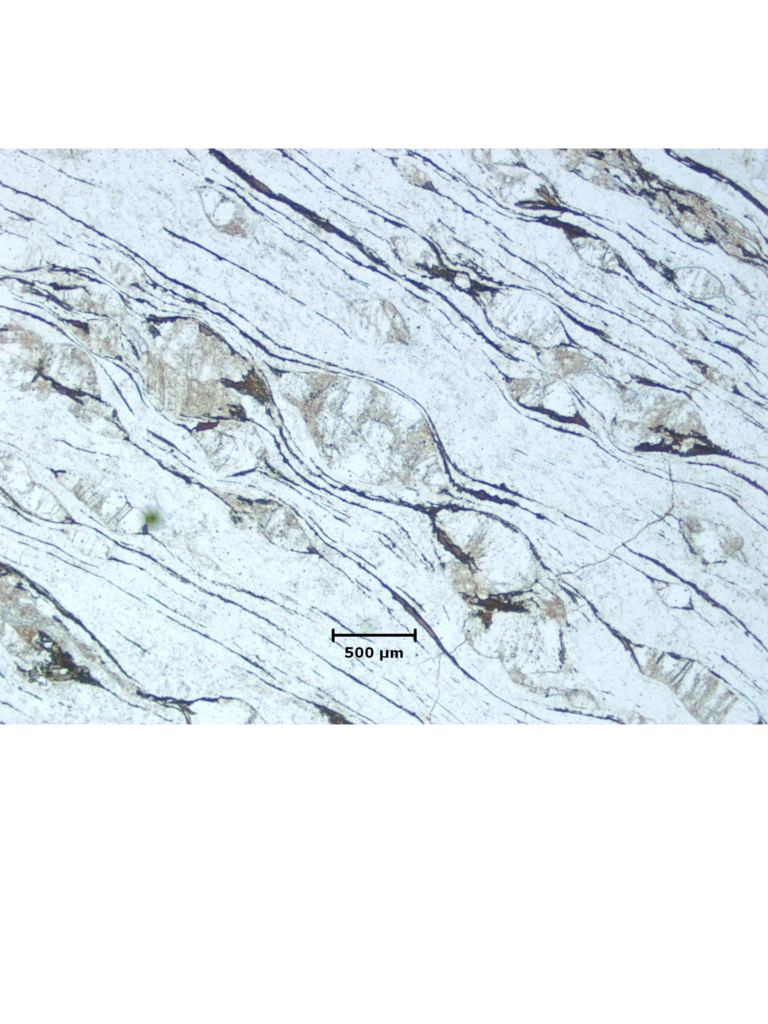
Rheology of polyphase rocks
We are carrying out a systematic investigation of the microstructure of granitoids deformed under a range of conditions from the onset of plasticity in quartz at ~300°C to the onset of dry melting at ~750°C, from samples for which we can constrain the pressure, temperature, water content and finite strain with reasonable accuracy. We can determine the differential stress during deformation from the dynamically recrystallized grain sizes in the quartz and feldspar, and we can interpret the microstructure in terms of the active deformational mechanisms in both phases, the effect of chemical interactions and phase changes, and the way the deformational mechanisms have changed during deformation. These data can then be integrated with experimental data to provide a description of the bulk rheology of the continental crust, and how it varies with depth and tectonic setting.
About the Image
The image shows feldspar porphyroclasts in a matrix of dynamically recrystallized quartz. The bulk rheology of a mylonite like this depends on the rheologies of the two phases, their proportions, and the mechanical interactions between the two phases, which in turn depend on the microstructure.
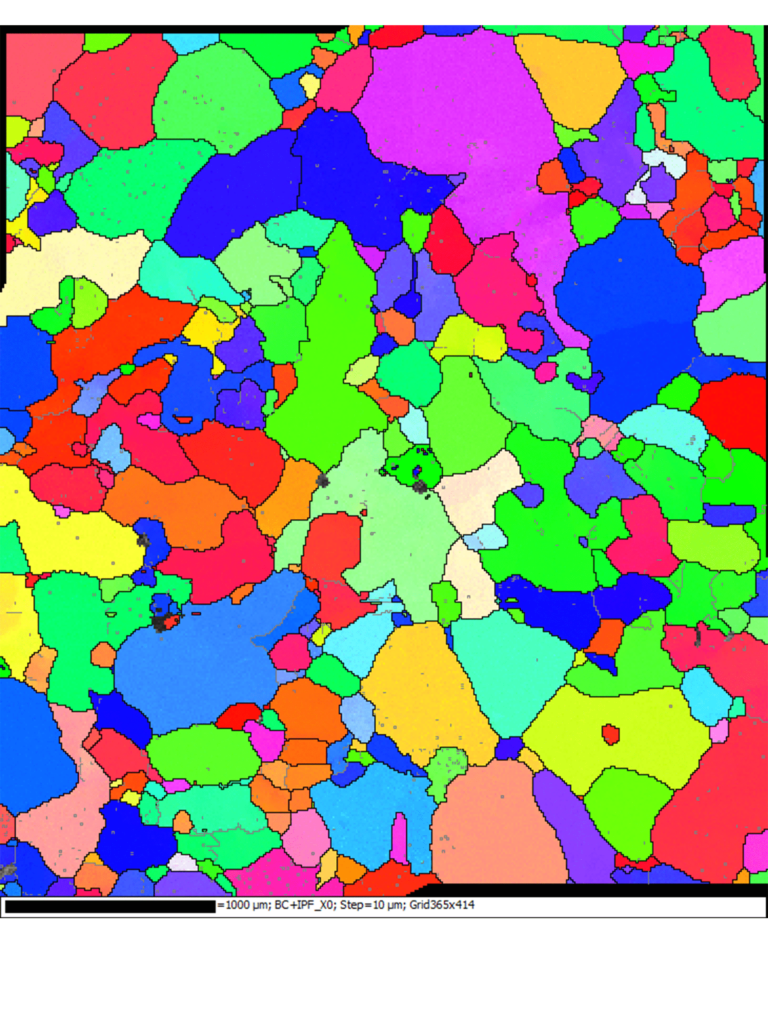
High-temperature microstructures in quartz and ice
Quartz and ice both exhibit distinctive microstructures when deformed at low stress and relatively high temperature, with large but variable grain sizes, amoeboid grain shapes with extensive grain-boundary lobes, low internal lattice distortion, and few subgrains. Strong crystallographic preferred orientations indicate dislocation creep. These microstructures (commonly referred to as GBM) reflect rapid grain-boundary migration and grain growth during deformation. They are difficult to reproduce experimentally in silicate minerals, and no correlation has been established between quantifiable aspects of the microstructure and deformational conditions such as differential stress. The purpose of this study is to investigate the effect of stress and temperature on GBM microstructures in ice, which is crystallographically analogous in several respects to quartz. This will contribute to our understanding of grain-size evolution in quartz and other minerals.
About the Image
The image here shows microstructure in ice experimentally deformed at close to the melting temperature (EBSD map)
Contact
Professor J. P. Platt
Department of Earth Sciences
University of Southern California
Los Angeles, CA90089-0740